Extending the lifetime of rail infrastructure by proper energy management
Posted: 28 January 2010 | | No comments yet
Current design philosophies of railway tracks are based on the conception of a railway system as a statically loaded system. This is directly translated into two classical principles: load spreading and stiffness reduction in downward direction. A ‘layered’ track design results in order to transfer the enormous wheel-rail contact stresses to the subsoil. However, the result is that track design is tuned on the short-term system response. In order to optimise the long-term behaviour of the system, the railway track must be conceived and designed as a dynamically loaded system, with due attention to the energy ‘streams’ through the system. Degradation processes are governed by energy conversion mechanisms, which are time-dependent. Therefore, the cyclic dynamic response of the system must be considered. This perspective will lead to decreased degradation rates, less maintenance, a better track quality permanence and lower Life Cycle Costs.
Current design philosophies of railway tracks are based on the conception of a railway system as a statically loaded system. This is directly translated into two classical principles: load spreading and stiffness reduction in downward direction. A ‘layered’ track design results in order to transfer the enormous wheel-rail contact stresses to the subsoil. However, the result is that track design is tuned on the short-term system response. In order to optimise the long-term behaviour of the system, the railway track must be conceived and designed as a dynamically loaded system, with due attention to the energy ‘streams’ through the system. Degradation processes are governed by energy conversion mechanisms, which are time-dependent. Therefore, the cyclic dynamic response of the system must be considered. This perspective will lead to decreased degradation rates, less maintenance, a better track quality permanence and lower Life Cycle Costs.
Current design philosophies of railway tracks are based on the conception of a railway system as a statically loaded system. This is directly translated into two classical principles: load spreading and stiffness reduction in downward direction. A ‘layered’ track design results in order to transfer the enormous wheel-rail contact stresses to the subsoil. However, the result is that track design is tuned on the short-term system response. In order to optimise the long-term behaviour of the system, the railway track must be conceived and designed as a dynamically loaded system, with due attention to the energy ‘streams’ through the system. Degradation processes are governed by energy conversion mechanisms, which are time-dependent. Therefore, the cyclic dynamic response of the system must be considered. This perspective will lead to decreased degradation rates, less maintenance, a better track quality permanence and lower Life Cycle Costs.
Design philosophies of railway infrastructure date back to the origination of the railways. The efficiency of rail transport is based on the very low friction between vehicle and vehicle carrier, which enables a very efficient use of the energy needed for displacement. Early trains had a relatively high weight and low speed. Therefore, apart from providing guidance and stability, load spreading was a key issue in the design of railway tracks. On a conceptual level, the railway track design was, and is, for that reason a static one, and historically a job that is being done by civil engineers. The static approach is being reflected in two principles that determine the construction of a railway: load spreading or increase in contact area at the interface between the components, and stiffness reduction of components, both in downward direction. The result is a ‘layered’ design: rail, railpads, sleepers, ballast, subgrade and ground. Both principles aim at a reduction of the extremely high wheel-rail contact stresses, which are amongst the highest ones known in engineering, to such a level that they do not cause permanent deformation in each consecutive track layer and no settlement of the soil.
Modern railway systems, and especially high-speed lines, are no longer predominantly statically loaded systems. This ‘shift’ is due to two reasons, which did not play an important role in the early times of the railways. Firstly, the effect of speed has become such that the track response may include dynamic effects, especially in countries with soft soils. One may think of delta or coastal areas with soft deposits. Since those areas are ‘flat’, they are often attractive for constructing high-speed network connections. In these areas, the train speed may reach critical wave velocities of ground layers in the track substructure, yielding significant dynamic amplifications of the track response to moving axle loads. Secondly, the rail surface is not a straight line. Geometrical track irregularities give rise to a dynamic response of the train-track system, and this is even the case for conventional railway lines with reduced speeds.
The above has a significant consequence for Life Cycle Cost analysis, track deterioration and the efficiency of track maintenance. When considering the railway from the traditional static viewpoint, deterioration, a time-dependent phenomenon, does not play a role. The track is being designed such that in each of its consecutive layers, the maximum stress under train loading does not exceed the elastic yield stress of the material. An exception occurs in the wheel-rail interface, where initial plasticity and work hardening are allowed for in the top layer of the rail during the first stages of wheel loading. In this way, damage, which is an instantaneous material overloading, is avoided. We may conclude that the traditional track design is focused on short-term system behaviour.
Is such a design efficient from the viewpoint of long-term performance? In order to answer this question, the railway system needs to be considered as a dynamically loaded system: the time dimension enters into consideration. This is necessary because all degradation mechanisms are basically dissipation processes of mechanical power as a function of time, affecting constitutive properties. One may illustrate this with a simple energy balance, considering a spatially bounded unit length of railway track, travelling along with the train. The power input into this volume is the electrical power that the train gets from the power supply. This energy is used partly for train motion (accelerating or decelerating, overcoming of rolling resistance); the remaining part of the energy is either dissipated within the volume or gives an energy flux over its boundaries. The first part is converted into heat or causes internal degradation. The second part leaves the system in the form of noise and/or vibration, often giving hindrance. Energy being improperly transferred into heat damages the material of the considered component. The long-term system response is therefore governed by the cyclic dynamic response: accumulation and saturation of plastic deformation, crack initiation and propagation, accumulation of material damage on a micro-scale and so on. This process is illustrated in Figure 1.
![Figure 1 Figure1: Mechanical power emission from an irregular wheel-rail contact through wave guides. The moving wheel-rail contact can be considered as a moving point source. Energy will redistribute over the system in space and time as a function of the geometrical and constitutive properties of the components. Energy of radiated waves can only be dissipated if components are designed (or added) to fullfill this function. ‘Unproperly’ dissipated energy causes long-term degradation. Therefore, dominant waveguides in the system should not be purely elastic, but visco-elastic[1]](http://www.globalrailwayreview.com/wp-content/uploads/Fig1.jpg)
![Figure 1 Figure1: Mechanical power emission from an irregular wheel-rail contact through wave guides. The moving wheel-rail contact can be considered as a moving point source. Energy will redistribute over the system in space and time as a function of the geometrical and constitutive properties of the components. Energy of radiated waves can only be dissipated if components are designed (or added) to fullfill this function. ‘Unproperly’ dissipated energy causes long-term degradation. Therefore, dominant waveguides in the system should not be purely elastic, but visco-elastic[1]](http://www.globalrailwayreview.com/wp-content/uploads/Fig1.jpg)
Figure1: Mechanical power emission from an irregular wheel-rail contact through wave guides. The moving wheel-rail contact can be considered as a moving point source. Energy will redistribute over the system in space and time as a function of the geometrical and constitutive properties of the components. Energy of radiated waves can only be dissipated if components are designed (or added) to fullfill this function. ‘Unproperly’ dissipated energy causes long-term degradation. Therefore, dominant waveguides in the system should not be purely elastic, but visco-elastic1
It is obvious from the previous that track irregularities should be minimised over the whole wavelength spectrum. To put it simply: there are wavelengths that cannot be perceived in the running train, but are perceived in or by the track, and precisely these waves are most damaging to the track. This is an important conclusion for rail infra managers. When a train runs at a constant speed over a perfectly smooth and homogeneous track, it only needs a constant and minimum power in order to overcome the ‘drag’ resulting from rolling resistance and finite track stiffness. There are no energy losses in what could be called ‘dynamic drag’. Another important consequence in the field of energy management is often neglected: the energy consumption of running trains can be significantly reduced by reducing track irregularities. It should be kept in mind that the same amount of energy that yields, for instance, vibration hindrance or a track settlement on a longer term is originally supplied via the overhead wire. Inclusion of the short wavelengths (<5m) in track geometry assessment has not always been common practice, because these waves do not play a role in ride and passenger comfort assessment. It is however a must from the viewpoint of reduction of degradation and maintenance. Especially since the privatisation of the European railways, with a separate exploitation of rail infra and rail transportation, the awareness of the key role of shorter wavelengths in track geometry assessment is growing. The Dutch rail infra manager ProRail adopted a cyclic rail grinding programme on its network with the aim of combatting the short waves2.
What is the performance of a conventional ballasted track concept, if it is briefly assessed from the discussed dynamic viewpoint? This question is of major importance for high-speed lines, which have high possession times and need a high reliability and optimum comfort. It is, in view of the current expansion of the European high-speed network, therefore highly relevant. The assessment methodology is illustrated in Figure 2 with a qualitative example. In this figure, the earlier defined convective track volume is being considered. The power input into the track follows from the track irregularity combined with the train speed and yields the dynamic excitation of the train-track system. This calculation yields a spectrum. Part of this spectrum is being dissipated into heat, whereas the remaining part is either being dissipated via improper modes, yielding degradation, or radiated from the volume as noise and vibration. It is obvious from the figure that only in the frequency band influenced by the railpads some proper conversion into heat takes place. A limitation is given here by the fact that heating (or even burning) of railpad material should not compromise its other functionality: providing elasticity. In the remaining frequency bands, proper conversion mechanisms are lacking. This is a major cause of track geometry deterioration on ballasted tracks, which is often observed to start from short-wave irregularities, such as insulated or dipped joints, bad welds, expansion joints, transitions in switches and crossings, severe corrugations, severe squats etc. These short irregularities yield a relatively significant contribution to the input power spectrum of the track which cannot be dissipated properly into heat.
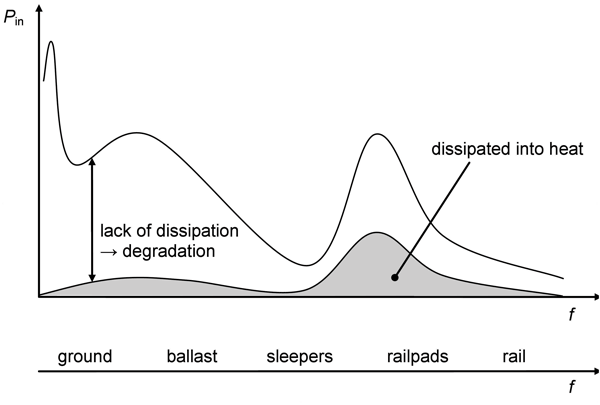
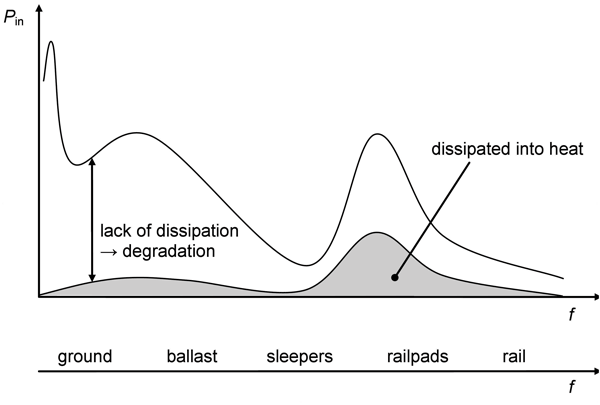
Figure 2: Mechanical power input spectrum into the track: partial conversion into heat and residual input leading to degradation and emission of noise and vibration
Experiments in European countries have confirmed the mechanisms explained in the previous and the lack of awareness of dynamics coupled to long-term considerations in track design. Field tests by ÖBB in Austria have shown that in regions with a highly dynamic loading regime such as switches and crossings, indeed the application of elastic undersleeperpads reduces the maintenance necessity (tamping, alignment) with in general a factor three3. This effect is generally being ascribed to better contact properties and a better development of elasticity through the track cross-section. However, a major effect is also due to energy dissipation, and this is often ignored. Without an appropriate layer between sleepers and ballast, the high-frequency contribution to the mechanical power input spectrum into the track reaches the ballast layer without being dissipated, causing very rapid degradation (in the form of grain refinement, surface smoothing, permanent deformation of the ballast layer). This problem was not that severe with the traditional wooden sleepers. Stated alternatively: the properties of a dominant waveguide in the system have been changed from almost purely elastic to visco-elastic by adding undersleeperpads in the layered track.
It can be concluded that railway track design needs to be reconsidered from a dynamic viewpoint, additionally to the existing viewpoint. Accounting for proper energy dissipation mechanisms throughout the whole frequency regime will allow for much lower degradation rates, extended maintenance intervals, a better quality permanence over time with higher safety and comfort levels. This can be translated via LCC analysis to a much better cost efficiency for railway administrators. It is quite remarkable that current European standards and regulations, apart from stiffness requirements, do not specify any damping requirements for track components. This is a direct result from the static track design perspective, and indirectly from the fact that the context of railway design is historically dominated by civil engineers and not mechanical engineers. It is a major challenge, and future investigations and developments need to address this issue properly.
References
- M.J.M.M. Steenbergen, Wheel-Rail Interaction at Short-Wave Irregularities, Ph.D. Thesis, Delft University of Technology, Delft, the Netherlands, 2008.
- W. Schoech, R. Heyder, R. Dollevoet, Specific railhead profiles to control rolling contact fatigue – design and maintenance, the European approach, Proc. of the 8th Int. Conf. on Contact Mechanics and Wear of Rail/Wheel Systems (CM2009), Firenze, Italy, September 15-18, 2009.
- Getzner, Erfahrungen mit besohlten Schwellen und Lösungen für Weichen, Conference, Schwarzenberg, Austria, November 14-16, 2007 (on CD-Rom).
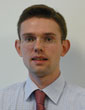
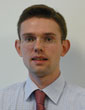
Michaël Steenbergen
About the author
Michaël Steenbergen is employed by Delft University of Technology as an academic researcher in the field of Railway Engineering. His research themes cover dynamic train-track interaction with a special focus on short defects at the interface, train-induced soil vibrations and track deterioration issues. Besides, he is involved in research and development with industrial parties, consultancy and in standardisation work for railway administrators. Since 2008 he holds a PhD cum laude from Delft University of Technology. He has written many publications on railway-related topics in scientific journals.